![]() |
Drug Target Insights 2023; 17: 39-44 ISSN 1177-3928 | DOI: 10.33393/dti.2023.2574 ORIGINAL RESEARCH ARTICLE |
![]() |
Treatment with levosimendan in an experimental model of early ventilator-induced diaphragmatic dysfunction
ABSTRACT
Introduction: Mechanical ventilation (MV) is a life-saving approach in critically ill patients. However, it may affect the diaphragmatic structure and function, beyond the lungs. Levosimendan is a calcium sensitizer widely used in clinics to improve cardiac contractility in acute heart failure patients. In vitro studies have demonstrated that levosimendan increased force-generating capacity of the diaphragm in chronic obstructive pulmonary disease patients. Thus the aim of this study was to evaluate the effects of levosimendan administration in an animal model of ventilator-induced diaphragmatic dysfunction (VIDD) on muscle contraction and diaphragm muscle cell viability.
Methods: Sprague-Dawley rats underwent prolonged MV (5 hours). VIDD+Levo group received a starting bolus of levosimendan immediately after intratracheal intubation and then an intravenous infusion of levosimendan throughout the study. Diaphragms were collected for ex vivo contractility measurement (with electric stimulation), histological analysis and Western blot analysis. Healthy rats were used as the control.
Results: Levosimendan treatment maintained an adequate mean arterial pressure during the entire experimental protocol, preserved levels of autophagy-related proteins (LC3BI and LC3BII) and the muscular cell diameter demonstrated by histological analysis. Levosimendan did not affect the diaphragmatic contraction or the levels of proteins involved in the protein degradation (atrogin).
Conclusions: Our data suggest that levosimendan preserves muscular cell structure (cross-sectional area) and muscle autophagy after 5 hours of MV in a rat model of VIDD. However, levosimendan did not improve diaphragm contractile efficiency.
Keywords: Diaphragm contractility, Levosimendan, Mechanical ventilation, Muscle fiber size, Ventilator-induced diaphragmatic dysfunction
Received: February 11, 2023
Accepted: March 29, 2023
Published online: April 13, 2023
Corresponding author:
Giacomo Bellani & Emanuele Rezoagli
School of Medicine and Surgery
University of Milano-Bicocca
via Cadore 48, 20900
Monza (MB) - Italy
giacomo.bellani1@unimib.it; emanuele.rezoagli@unimib.it
Drug Target Insights - ISSN 1177-3928 - www.aboutscience.eu/dti
© 2023 The Authors. This article is published by AboutScience and licensed under Creative Commons Attribution-NonCommercial 4.0 International (CC BY-NC 4.0).
Commercial use is not permitted and is subject to Publisher’s permissions. Full information is available at www.aboutscience.eu
Introduction
Mechanical ventilation (MV) is a life support technique used in critically ill patients. However, prolonged MV is also associated with numerous potential complications including a rapid impairment of both lungs (i.e., ventilator-induced lung injury – VILI) and diaphragm (i.e., ventilator-induced diaphragmatic dysfunction – VIDD). MV can also exacerbate a preexisting VILI (1-3). It is also associated with adverse effects on multiple aspects of diaphragmatic structure and function (VIDD) (4). The detrimental impact of prolonged MV on the diaphragm is strictly correlated to problems in weaning patients from the ventilator (5). The incidence level can reach 35% of patients exposed to prolonged MV, with subsequent increase in morbidity and mortality (4,6,7). Furthermore, body composition indexes are known to predict outcome – such as mortality and MV duration itself – in critically ill patients (8). The first evidence on the correlation between MV and VIDD was published almost 35 years ago, in which a study of infant and neonates suggested that MV predisposes diaphragm fibers to atrophy (9). Thenceforth numerous experimental and clinical studies documented that prolonged MV induces a rapid muscular atrophy and contractile dysfunction (10).
Skeletal muscle is one of the most significant muscle tissues in the body. Thousands of muscle fibers are encased in connective tissue sheaths to form each skeletal muscle. The size of skeletal muscle fiber is dependent on the balance between the protein synthesis and protein degradation (11,12). Animal studies have demonstrated that protein synthesis declines rapidly within the first 6 hours of MV and remains depressed during the next 12 hours (11). At the same time, the prolonged MV increases the activity of all major proteolytic systems: macroautophagy, calpains, caspases and the ubiquitin-proteasome systems. Moreover prolonged MV results in oxidative damage to the diaphragm – reactive oxygen species and their derivatives have a significant impact on skeletal muscle contractile function (13).
Prolonged MV results in less efficient calcium activation of diaphragm fibers most likely due to oxidative modification of diaphragm contractile proteins (14). Levosimendan is a calcium sensitizer, first described in 1995, that is administered to enhance cardiac contractility in patients with acute heart failure (15,16). In addition, levosimendan improves respiratory muscle function in healthy subjects and patients with chronic obstructive pulmonary disease (COPD) (17), through the improvement of calcium sensitization of the contractile proteins (18). Moreover, it reduces inflammation and oxidative stress (19). A recent study also demonstrated that levosimendan dampens nitrosative stress in the diaphragm of mechanically ventilated endotoxemic mice (20).
Thus, we administered levosimendan to an in vivo model of VIDD to determine if it could alleviate the negative impacts of MV. Therapeutic effects were determined by measuring muscle contraction and diaphragm muscle viability. The aim of the study was to evaluate the effect of the treatment of levosimendan in a rat model on VIDD on:
- the preservation of diaphragm muscle structure;
- the level of autophagy activation in the diaphragm;
- the diaphragm muscular contractile function.
Materials and methods
Animals
Male Sprague-Dawley rats (250-300 g; Envigo RMS S.r.l., Udine, Italy) were used in this study. Animals were housed two per cage in a limited access animal facility, with the room temperature at 20 ± 2°C and the relative humidity set at 55 ± 10%. Artificial lighting provided a 12-hour light/12-hour dark (7 am.–7 pm) cycle. The general condition of the animals before the experiment was assessed daily. The care and husbandry of animals were in conformity with the institutional guidelines in compliance with Italian and European laws and policies. The animal study was reviewed and approved by the Italian Ministry of Health (773/2018-PR) and by the Animal Care Unit of the University of Milano-Bicocca, Monza, Italy. In full respect of the reduction principle of the 3Rs (21), the number of animals/groups was selected to obtain reliable results and enough biological samples to perform the analysis planned.
Experimental protocol
The experimental design is composed of three experimental group of rats:
– Healthy: non-anesthetized and spontaneously breathing rats;
– VIDD: anesthetized and mechanically ventilated rats without any pharmacological treatment;
– VIDD+Levo: anesthetized and ventilated rats with levosimendan infusion.
Rats were anesthetized with ketamine (100 mg/kg) and xylazine (4 mg/kg), orotracheally intubated and ventilated for 5 hours (Harvard Inspira; tidal volume: 10 mL/kg; respiratory rate: 80/min; positive end-expiratory pressure [PEEP]: 2-2.5 cmH2O; fraction of inspired oxygen [FiO2: 0.5]). Anesthesia and paralysis were maintained throughout the experiment by infusion in the right femoral artery of propofol (13 mg/kg/h) and ketamine (5 mg/kg/h) and in the right jugular vein of rocuronium bromide (1.5 mg/kg/h) and Ringer acetate (1.8 mL/h). Airway pressure and hemodynamic parameters were monitored through pressure transducers in ventilator and at the arterial catheter. A recruitment maneuver (30 cmH2O for 10 sec) was performed every 60 minutes, being the plateau pressure, and respiratory system static compliance was measured every hour. Rats belonging to the treatment group (VIDD+Levo) received a dose (24 µg/kg) of levosimendan (Simdax; Orion Pharma) into the tail vein at the beginning of the experiment and then a maintenance of the treatment was achieved by an infusion in the left jugular vein of levosimendan (0.2 µg/kg/min) (22).
Pulmonary function
For the lung mechanical properties, a pressure to volume (PV) curve was calculated. After a recruitment maneuver, five steps of 0.5 mL inspiratory volumes (i.e., total 2.5 mL) were delivered into the lungs. For each step, the plateau pressure was recorded to calculate the static compliance.
Diaphragmatic contractile function
Diaphragmatic contractile function was measured as already described (23), briefly a diaphragm strip was mounted into a tissue bath and, through an electric stimulator, the peak tetanic tension and the force-frequency relationship were evaluated.
Histological analysis: muscular fibers cross-sectional area
A section from the right and one from the left hemidiaphragm tissue was used for histology analysis. The cross-sectional area (CSA) of the muscular fiber was determined by manually tracing the cell contour on digitized images from hematoxylin and eosin-stained frozen section. The CSA of at least 150 fibers per diaphragm were then averaged.
Western blot analysis
Another section of the diaphragm excised at the sacrifice was immediately frozen in liquid nitrogen and stored at −80°C. The cytoplasmic extraction was prepared using an NE-PER Nuclear Cytoplasmic Extraction Reagent kit (Pierce, Rockford, IL, USA) according to the manufacturer’s instruction. Equal amounts of the protein concentrations were quantified by the bicinchoninic acid assay (BCA assay; Pierce, Rockford, IL, USA), and each sample was analyzed according to standard Western blotting protocols. The following antibodies were used: atrogin (AP2041; ECM Biosciences, Versailles, KY, USA) and LC3B (light chain 3, isoform B II, 2775; Cell signaling technology, Danvers, MA, USA). The antibody α-tubulin (#2125; Cell Signaling, Danvers, MA, USA) was used as the reference of internal control.
Statistical analysis
Data are expressed as mean ± standard error of the mean (SEM). Differences in variances between groups were assessed by one-way analysis of variance (ANOVA). Post hoc comparisons were performed by Benjamini, Krieger and Yekutieli. Differences in physiological variables between VIDD and VIDD+Levo were performed by unpaired t-test. p-Values < 0.05 were considered as statistically significant (two-tailed). Statistical analysis was performed by GraphPad Prism (version 8.4.2). Some histologic and Western blot analyses could not be performed in some animals because of technical problems.
Results
Systemic response
No rats died during the experiments. In terms of body weight, no differences were found between groups (healthy: 310 ± 6, VIDD: 300 ± 6, VIDD + Levo: 295 ± 7 g; ANOVA p = 0.25). The mean arterial pressure (MAP) was similar between the test groups at the beginning of the experiment and after 3 hours of MV. At the end of the MV, rats without levosimendan treatment showed a significant lower MAP versus VIDD + Levo group, as demonstrated in Table I. The difference in MAP was not influenced by a difference in terms of fluid balance, since it was similar between two groups (VIDD: 18.6 ± 0.6 mL vs. VIDD + Levo: 19.9 ± 1.3 mL, p = 0.31). Regarding the respiratory function, no differences were found between ventilated groups in oxygenation nor in respiratory system static compliance.
Histological analysis
Levosimendan treatment seemed to induce a protection in muscle fiber size from cellular atrophy induced by MV. As shown in Figure 1, MV affects the cross-sectional fiber area in VIDD animals if compared to healthy rats. VIDD+Levo group had fiber diameters like unventilated animals.
VIDD | VIDD + Levo | p-Values | ||
---|---|---|---|---|
Mean arterial pressure(mm Hg) | Start | 90 ± 5 | 91 ± 11 | 0.93 |
3 hours | 94 ± 6 | 97 ± 9 | 0.78 | |
End | 66 ± 6 | 89 ± 10 | 0.04* | |
Heart rate(/min) | Start | 254 ± 10 | 285 ± 16 | 0.10 |
3 hours | 329 ± 12 | 329 ± 6 | 0.95 | |
End | 306 ± 12 | 330 ± 9 | 0.15 | |
PaO2/FiO2 | End | 476 ± 48 | 529 ± 13 | 0.25 |
Respiratory system static compliance (mL/cmH2O) | End | 0.34 ± 0.01 | 0.32 ± 0.01 | 0.38 |
All parameters were measured during mechanical ventilation. VIDD: MV + no treatment (n = 12); VIDD+Levo: MV + levosimendan treatment (n = 8).
MV = mechanical ventilation; VIDD = ventilator-induced diaphragmatic dysfunction.
*p < 0.05.
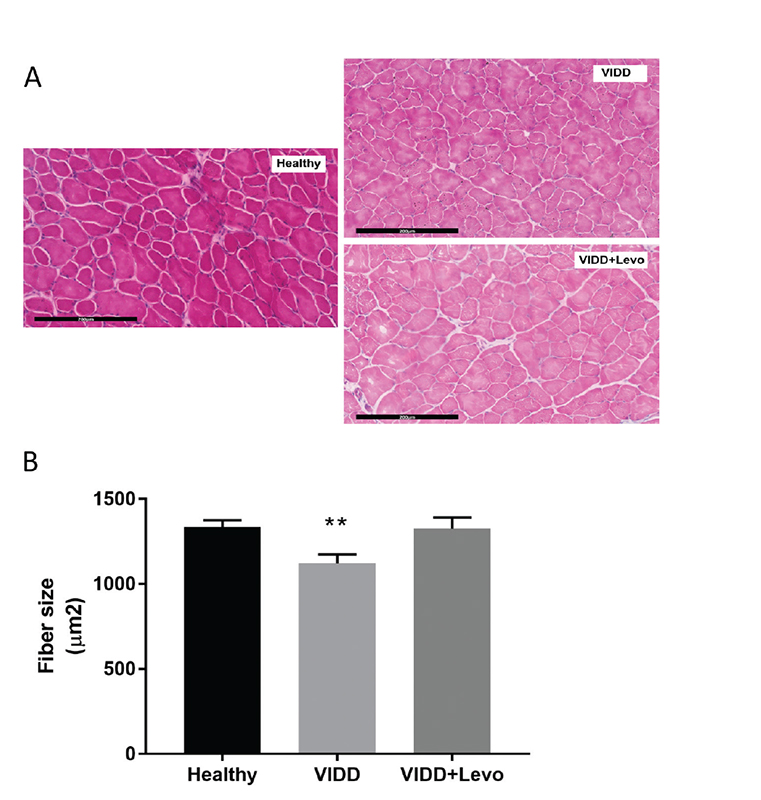
Fig. 1 - Diaphragm fiber size. Cross-sectional diaphragmatic fiber size in histological slice. A) Histological images, hematoxylin-eosin staining, 200×, scale bar = 200 µm. B) ANOVA p < 0.01. Benjamini, Krieger and Yekutieli post hoc test: p = 0.004 vs. healthy and p = 0.01 vs. VIDD+Levo. Healthy: no surgical interventions, no MV, and no treatment (n = 8); VIDD: surgical intervention + MV and no treatment (n = 10); VIDD+Levo: surgical intervention + MV + levosimendan treatment (n = 8). ANOVA = analysis of variance; MV = mechanical ventilation; VIDD = ventilator-induced diaphragmatic dysfunction.
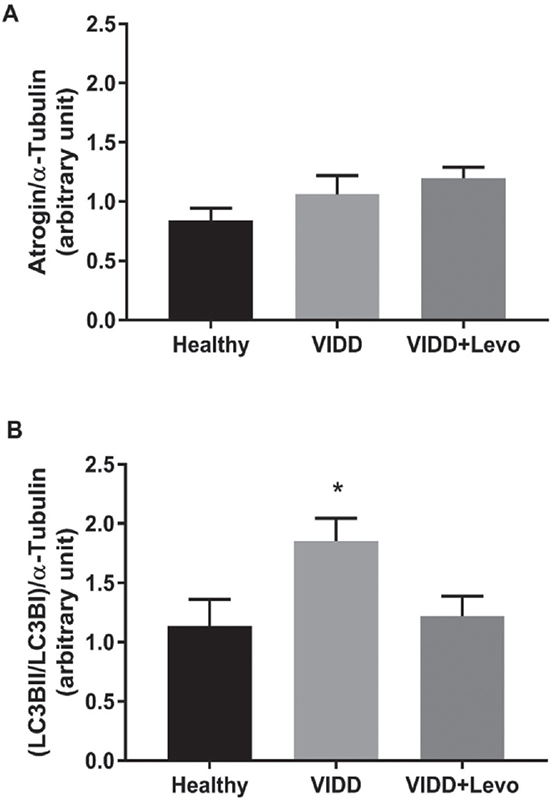
Fig. 2 - Western blot analysis. Atrogin and LC3BII/LC3BI levels on diaphragm tissue. A) ANOVA p = 0.21. B) ANOVA p = 0.03. Benjamini, Krieger and Yekutieli post hoc test: p = 0.02 vs. healthy and p = 0.04 vs. VIDD+Levo. Healthy: no surgical interventions, no MV, and no treatment (n = 8); VIDD: surgical intervention + MV and no treatment (n = 11); VIDD+Levo: surgical intervention + MV + levosimendan treatment (n = 8). ANOVA = analysis of variance; MV = mechanical ventilation; VIDD = ventilator-induced diaphragmatic dysfunction.
Western blot analysis
Western blot analysis (Fig. 2) on levels of proteins involved in muscle protein degradation (atrogin) did not reveal any statistical difference between groups. Proteins implicated in autophagy and autophagy-related processes (LC3BI and LC3BII) were significantly higher in the VIDD group, if compared to Healthy and VIDD+Levo groups, while these proteins did not differ in the VIDD+Levo group as compared with the Healthy group.
Diaphragm contractile dysfunction
After 5 hours of MV, rats that underwent ventilation (VIDD and VIDD+Levo) showed a significant reduction in diaphragmatic contractility in response to in vitro electric stimulation, in comparison to unventilated animals. As demonstrated in Figure 3A, increasing the frequency of stimulation the diaphragmatic muscle strip of ventilated rats generated less force than the healthy diaphragm. Levosimendan treatment did not affect the diaphragmatic contractility. The analysis of peak tetanic tension unveiled that ventilated rats (with or without levosimendan treatment) showed a similar diaphragmatic muscular contractility (Fig. 3B).
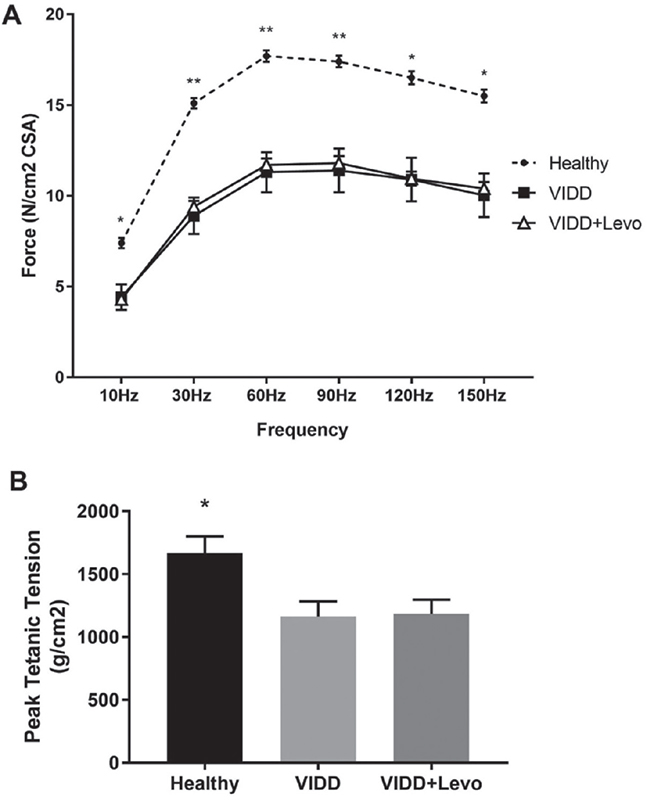
Fig. 3 - Diaphragm contractility. Force-frequency relationship (panel A) and peak tetanic tension (panel B) measured on diaphragmatic strips with electric stimulator at the end of the experiment. A) ANOVA *p < 0.05, **p < 0.01. Benjamini, Krieger and Yekutieli post hoc test: 10 Hz p = 0.03 vs. VIDD and vs. VIDD+Levo, 30 Hz p = 0.001 vs. VIDD and p = 0.004 vs. VIDD+Levo, 60 Hz p = 0.002 vs. VIDD and p = 0.005 vs. VIDD+Levo, 90 Hz p = 0.004 vs. VIDD and p = 0.01 vs. VIDD+Levo, 120 Hz p = 0.006 vs. VIDD and p = 0.01 vs. VIDD+Levo, 150 Hz p = 0.007 vs. VIDD and p = 0.002 vs. VIDD+Levo. B) ANOVA p < 0.05. ANOVA = analysis of variance; VIDD = ventilator-induced diaphragmatic dysfunction. B) ANOVA p < 0.05. Benjamini, Krieger and Yekutieli post-hoc test: p = 0.007 vs VIDD, p = 0.01 vs VIDD+Levo. Healthy: no surgical interventions, no MV, and no treatment (n = 8); VIDD: surgical intervention + MV and no treatment (n = 12); VIDD+Levo: surgical intervention + MV + Levosimendan treatment (n = 8).
Discussion
In this experimental model of VIDD, after 5 hours of MV in a rat model of VIDD as compared to control, our data suggest that the administration of levosimendan:
- preserves the diaphragm muscular cell structure;
- reduces autophagy-implicated proteins of the diaphragm muscle cells;
- does not affect diaphragm contractile function.
Levosimendan is a calcium-sensitizing inodilator widely used in the management of acutely decompensated chronic heart failure from over 20 years. In addition, it has been evaluated in a variety of clinical settings for both cardiac and non-cardiac disease (24,25). One possible area of investigation is respiratory muscle dysfunction, because, in addition to cellular atrophy, reduced calcium sensitivity of contraction causes respiratory muscle weakness (26). In vitro studies on isolated muscle fibers showed that levosimendan increased force-generating capacity of diaphragm from COPD and non-COPD patients by improving calcium sensitivity of force generation (17). Clinical studies have produced mixed results, the same investigators discovered that levosimendan increased in vivo diaphragmatic contractile efficiency in healthy participants (27), while it had no effect on diaphragm function in intensive care unit (ICU) patients (28). The role of levosimendan as potential therapeutic intervention of diaphragm dysfunction is an open field of current pharmacological research for VIDD in addition to the one for VILI because of the need of MV in the presence of severe respiratory failure (3,29).
In this study we evaluated the effects of levosimendan treatment in preclinical in vivo rodent model of VIDD. We have previously demonstrated that the model resembles the main features of diaphragmatic dysfunction (22). As levosimendan has a short half-life of 1 hour, a bolus of 24 µg/kg was immediately administered after orotracheal intubation and MV. It is well known that the use of a bolus dose should be avoided due to the risk of hypotension (30), but the animals in this study were healthy at the beginning of the study with physiological hemodynamics, as shown in Table I. In our experimental design, levosimendan treatment, rather than inducing hypotension, maintained higher levels of MAP as compared to untreated animals at the end of experiment (Tab. I).
The diaphragmatic contraction was evaluated by in vitro electric stimulation after 5 hours of MV: both ventilated groups showed a significant reduction in muscular force compared to healthy control rats (Fig. 3A, B). Levosimendan treatment did not improve diaphragm contraction, maybe due to the bolus dose used in the study: 24 vs. 40 µg/kg, which was used in similar investigations (27). However, histological analysis of muscular diaphragmatic fiber revealed that levosimendan administration preserved significant cellular diameter from atrophy. Prolonged MV led to a reduction in cross-sectional muscular fiber area of 16% (Fig. 1), whereas levosimendan-treated rats showed conserved cellular structure when compared to the VIDD group. The levels of atrogin, a protein belonging to the ubiquitin-proteasome system of proteolysis, did not differ between groups (Fig. 2), whereas microtubule-associated protein light chain 3 I and II (LC3BI and II), autophagosome marker, were significantly modified by MV. It has been previously reported that prolonged MV can lead to upregulation of atrogin and LC3B (4,31). We hypothesize that, in our experimental protocol, the hours of MV should influence the protein expression, maybe 5 hours MV were not sufficient to stimulate atrogin production.
This study has some limitations that should be acknowledged. First, the duration of MV (5 hours) potentially did not allow enough time to evaluate all the parameters (such as atrogin levels). However, the purpose of this study was to determine the early effects of levosimendan. Early administration ensured that possible side effects on hemodynamics were avoided. It has been previously demonstrated that MV can have detrimental effects on muscle fibers. Therefore, future studies will identify more specifically the different types of muscle fibers after MV with and without treatment. Second, we decided to administer the dose of levosimendan used routinely in the clinical setting (decompensated heart failure). We cannot exclude that the use of a higher dose of levosimendan might provide a benefit in terms of diaphragmatic contraction. A dose-response study may be a useful potential step to further explore the role of levosimendan during VIDD. Third, during the MV period, all rats received a continuous infusion of rocuronium bromide that may probably affect the diaphragmatic muscle contraction that was assessed ex vivo after animal euthanasia. Indeed, it is known that, simultaneously with MV, some drugs – such as neuromuscular blocking agents – may worsen the diaphragm dysfunction, albeit data on their effects on diaphragm are not debated (32-35).
In conclusion, in an experimental animal model of VIDD, levosimendan prevented autophagy allowing to preserve diaphragm muscular cellular structure as compared with VIDD untreated group. However, levosimendan did not improve the diaphragm contractile efficiency.
Disclosures
Conflict of interest: The authors declare that the research was conducted in the absence of any commercial or financial relationships that could be construed as a potential conflict of interest.
Funding/Support: We acknowledge that this research was partially supported by the Italian Ministry of University and Research (MIUR), Department of Excellence project PREMIA (PREcision MedIcine Approach: bringing biomarker research to clinic). Dr. Emanuele Rezoagli was supported by the Bicocca Starting grant 2020 from the University of Milano-Bicocca with the project titled: “Functional Residual Capacity Assessment using a Wash-In/Wash-Out technique based on a fast mainstream O2 Sensor with nanofluorescenT geometry for severe lung injury (FAST) – COVID and beyond” and by the International Young Investigator Award 2018 from the European Society of Intensive Care Medicine (ESICM) with the project titled: “Role of the exhaled breath condensate as non-invasive monitoring of the lung inflammation during ARDS: a prospective cohort study.”
References
- 1. Dreyfuss D, Saumon G. Ventilator-induced lung injury: lessons from experimental studies. Am J Respir Crit Care Med. 1998;157(1):294-323. CrossRef PubMed
- 2. Rezoagli E, Laffey JG, Bellani G. Monitoring lung injury severity and ventilation intensity during mechanical ventilation. Semin Respir Crit Care Med. 2022;43(3):346-368. CrossRef PubMed
- 3. Horie S, McNicholas B, Rezoagli E, et al. Emerging pharmacological therapies for ARDS: COVID-19 and beyond. Intensive Care Med. 2020;46(12):2265-2283. CrossRef PubMed
- 4. Powers SK, Wiggs MP, Sollanek KJ, Smuder AJ. Ventilator-induced diaphragm dysfunction: cause and effect. Am J Physiol Regul Integr Comp Physiol. 2013;305(5):R464-R477. CrossRef PubMed
- 5. Gatti S, Abbruzzese C, Ippolito D, et al. Ultrasound versus computed tomography for diaphragmatic thickness and skeletal muscle index during mechanical ventilation. Diagnostics (Basel). 2022;12(11):2890. CrossRef PubMed
- 6. Vassilakopoulos T, Petrof BJ. Ventilator-induced diaphragmatic dysfunction. Am J Respir Crit Care Med. 2004;169(3):336-341. CrossRef PubMed
- 7. Pham T, Heunks L, Bellani G, et al. Weaning from mechanical ventilation in intensive care units across 50 countries (WEAN SAFE): a multicentre, prospective, observational cohort study. Lancet Respir Med. 2023 Jan 20:S2213-2600(22)00449-0. CrossRef PubMed. Epub ahead of print.
- 8. Giani M, Rezoagli E, Grassi A, et al. Low skeletal muscle index and myosteatosis as predictors of mortality in critically ill surgical patients. Nutrition. 2022;101:111687. CrossRef PubMed
- 9. Knisely AS, Leal SM, Singer DB. Abnormalities of diaphragmatic muscle in neonates with ventilated lungs. J Pediatr. 1988;113(6):1074-1077. CrossRef PubMed
- 10. Powers SK, Kavazis AN, Levine S. Prolonged mechanical ventilation alters diaphragmatic structure and function. Crit Care Med. 2009;37(10)(suppl):S347-S353. CrossRef PubMed
- 11. Shanely RA, Van Gammeren D, Deruisseau KC, et al. Mechanical ventilation depresses protein synthesis in the rat diaphragm. Am J Respir Crit Care Med. 2004;170(9):994-999. CrossRef PubMed
- 12. Tobin MJ, Laghi F, Jubran A. Narrative review: ventilator-induced respiratory muscle weakness. Ann Intern Med. 2010;153(4):240-245. CrossRef PubMed
- 13. Powers SK, Jackson MJ. Exercise-induced oxidative stress: cellular mechanisms and impact on muscle force production. Physiol Rev. 2008;88(4):1243-1276. CrossRef PubMed
- 14. Andrade FH, Reid MB, Westerblad H. Contractile response of skeletal muscle to low peroxide concentrations: myofibrillar calcium sensitivity as a likely target for redox-modulation. FASEB J. 2001;15(2):309-311. CrossRef PubMed
- 15. Pollesello P, Ovaska M, Kaivola J, et al. Binding of a new Ca2+ sensitizer, levosimendan, to recombinant human cardiac troponin C. A molecular modelling, fluorescence probe, and proton nuclear magnetic resonance study. J Biol Chem. 1994;269(46):28584-28590. Erratum in: J Biol Chem. 1995 Feb 10;270. 6.: 2880. CrossRef PubMed
- 16. Haikala H, Kaivola J, Nissinen E, Wall P, Levijoki J, Lindén IB. Cardiac troponin C as a target protein for a novel calcium sensitizing drug, levosimendan. J Mol Cell Cardiol. 1995;27(9):1859-1866. CrossRef PubMed
- 17. van Hees HW, Dekhuijzen PN, Heunks LM. Levosimendan enhances force generation of diaphragm muscle from patients with chronic obstructive pulmonary disease. Am J Respir Crit Care Med. 2009;179(1):41-47. CrossRef PubMed
- 18. Follath F, Cleland JGF, Just H, et al; Steering Committee and Investigators of the Levosimendan Infusion versus Dobutamine (LIDO) Study. Efficacy and safety of intravenous levosimendan compared with dobutamine in severe low-output heart failure (the LIDO study): a randomised double-blind trial. Lancet. 2002;360(9328):196-202. CrossRef PubMed
- 19. Sareila O, Korhonen R, Auvinen H, et al. Effects of levo- and dextrosimendan on NF-kappaB-mediated transcription, iNOS expression and NO production in response to inflammatory stimuli. Br J Pharmacol. 2008;155(6):884-895. CrossRef PubMed
- 20. Schellekens WJ, van Hees HW, Linkels M, et al. Levosimendan affects oxidative and inflammatory pathways in the diaphragm of ventilated endotoxemic mice. Crit Care. 2015;19(1):69. CrossRef PubMed
- 21. Díaz L, Zambrano E, Flores ME, et al. Ethical considerations in animal research: the principle of 3R’s. Rev Invest Clin. 2020;73(4):199-209. CrossRef PubMed
- 22. Innes CA, Wagstaff AJ. Levosimendan: a review of its use in the management of acute decompensated heart failure. Drugs. 2003;63(23):2651-2671. CrossRef PubMed
- 23. Zambelli V, Sigurtà A, Rizzi L, et al. Angiotensin-(1-7) exerts a protective action in a rat model of ventilator-induced diaphragmatic dysfunction. Intensive Care Med Exp. 2019;7(1):8. CrossRef PubMed
- 24. Papp Z, Agostoni P, Alvarez J, et al. Levosimendan efficacy and safety: 20 years of SIMDAX in clinical use. J Cardiovasc Pharmacol. 2020;76(1):4-22. CrossRef PubMed
- 25. Girardis M, Bettex D, Bojan M, et al. Levosimendan in intensive care and emergency medicine: literature update and expert recommendations for optimal efficacy and safety. J Anesth Analg Crit Care. 2022;2(1):1-22. CrossRef
- 26. Hooijman PE, Beishuizen A, de Waard MC, et al. Diaphragm fiber strength is reduced in critically ill patients and restored by a troponin activator. Am J Respir Crit Care Med. 2014;189(7):863-865. CrossRef PubMed
- 27. Doorduin J, Sinderby CA, Beck J, et al. The calcium sensitizer levosimendan improves human diaphragm function. Am J Respir Crit Care Med. 2012;185(1):90-95. CrossRef PubMed
- 28. Roesthuis L, van der Hoeven H, Sinderby C, et al. Effects of levosimendan on respiratory muscle function in patients weaning from mechanical ventilation. Intensive Care Med. 2019;45(10):1372-1381. CrossRef PubMed
- 29. Zambelli V, Rizzi L, Delvecchio P, et al. Hexarelin modulates lung mechanics, inflammation, and fibrosis in acute lung injury. Drug Target Insights. 2021;15:26-33. CrossRef PubMed
- 30. Nieminen MS, Buerke M, Cohen-Solál A, et al. The role of levosimendan in acute heart failure complicating acute coronary syndrome: a review and expert consensus opinion. Int J Cardiol. 2016;218:150-157. CrossRef PubMed
- 31. Schellekens WJ, van Hees HW, Vaneker M, et al. Toll-like receptor 4 signaling in ventilator-induced diaphragm atrophy. Anesthesiology. 2012;117(2):329-338. CrossRef PubMed
- 32. Dres M, Demoule A. Diaphragm dysfunction during weaning from mechanical ventilation: an underestimated phenomenon with clinical implications. Crit Care. 2018;22(1):73. CrossRef PubMed
- 33. Testelmans D, Maes K, Wouters P, et al. Rocuronium exacerbates mechanical ventilation-induced diaphragm dysfunction in rats. Crit Care Med. 2006;34(12):3018-3023. CrossRef PubMed
- 34. Testelmans D, Maes K, Wouters P, Powers SK, Decramer M, Gayan-Ramirez G. Infusions of rocuronium and cisatracurium exert different effects on rat diaphragm function. Intensive Care Med. 2007;33(5):872-879. CrossRef PubMed
- 35. Hraiech S, Forel JM, Papazian L. The role of neuromuscular blockers in ARDS: benefits and risks. Curr Opin Crit Care. 2012;18(5):495-502. CrossRef PubMed